By Stephen Eick
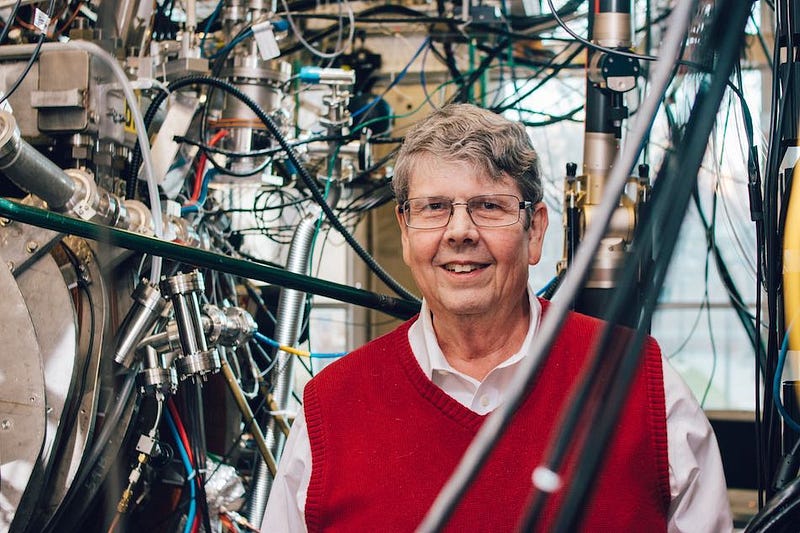
Energy is everything. Without energy, Earth would be a frozen hunk of rock meandering through space. Without energy, society would cease to exist as we know it today. Humans use water, the oil in the earth, the wind, the sun, and many other methods to generate this energy. Some methods harm our world while others are still quite inefficient. More exotic methods exist that show immense potential to solve both these problems. One in particular is the plasma fusion reactor. The pursuit of the plasma fusion reactor is one of the most complex endeavors ever undertaken by humans. By attempting to harness the power of nuclear fusion, we are attempting to generate energy in a method identical to our sun. Here at UW-Madison and across the world, researchers are working to make fusion energy a reality.
In order to truly appreciate the challenges involved and benefits to be gained from fusion energy, we need to take it slow. First, it’s necessary to clarify the difference between nuclear fission and nuclear fusion. Nuclear physics tells us that elements with very low atomic numbers such as hydrogen and very high atomic numbers such as uranium release the most energy when they undergo a nuclear reaction. However, the ways we extract energy from heavy and light elements are fundamentally different. Heavy atoms such as uranium can be cleaved into smaller pieces either by natural decay of the atom or by ramming the atoms with neutrons. This process is known as nuclear fission, which has held widespread popularity in our world for the last 70 years in both peaceful (power plants and medical isotope production) and proliferative (nuclear weapon stockpiling) positions. In short, nuclear fission splits an atom into smaller pieces.
Directly opposite to nuclear fission is nuclear fusion. Nuclear fusion is the process of melding atoms together. While nuclear fission uses heavy elements, nuclear fusion uses light elements. Energy is extracted when the fusing atoms change mass as dictated by the famous E=mc2 equation. The core concept behind nuclear fusion is to have atoms bump into and fuse with each other. For this to be accomplished, two forces need to be taken into account: electric repulsion, which pushes atoms away from each other, and the strong nuclear force, which holds the atom together. The strong nuclear force is much stronger than electric repulsion but only when the atoms are at very short distances from each other. For nuclear fusion to begin, electric repulsion must be overcome. The most common way to overcome electric repulsion is to heat the atoms to increase their kinetic energy past a specific threshold. However, the temperatures required range to millions of degrees, which makes this problem significantly more difficult; no material in existence can hold matter of such temperature extremes.
Heating particles to such high temperatures shifts the particles to the fourth state of matter known as plasma. Plasma is formed after heating a gas to very high temperatures. A key property of plasma is that it feels forces in electromagnetic fields. With this property in mind, researchers developed a nuclear fusion technique that shoots plasma around in a magnetically-confined ring. A device that uses this confinement technique is the donut-shaped tokamak. The tokamak, whose name comes from a Russian acronym, is the most developed fusion-confinement approach to date and is widely used for its good confinement properties and simple design.
Yet, the design of the tokamak has some limiting aspects. To understand why, imagine taking a Slinky and putting the two ends of it together. The formed shape is a donut made of helical lines. This is the required magnetic field structure for a tokamak. However, creating this structure requires two separate magnetic fields: one to produce the field going around the donut, and one to twist the field in a spiral. The tokamak requires an electric current to be driven through the plasma to produce this twisting field. As a result, this approach is inherently unstable and has a higher potential for the plasma to lose confinement. If the plasma escapes the magnetically-confined ring through this instability, the immense energy contained would lash outward, potentially destroying the fusion reactor.
To counteract these problems with the tokamak, a more advanced type of fusion reactor was developed. This reactor, known as a stellarator, uses unconventional configurations of magnets to achieve plasma confinement similar to the tokamak while eliminating the need to drive current through the plasma. This reduces the instability problems of tokamaks and allows for a more efficient confinement device. However, the stellarator introduces a new problem. The tokamak, with its symmetric donut shape, has symmetric magnetic fields, which are desirable. The classical stellarator, with its unconventional magnets, produces asymmetric magnetic fields, which introduces a whole host of new confinement problems.
It is this asymmetry problem that UW-Madison ECE Professor David Anderson has set out to solve. After receiving his PhD from UW-Madison, he continued to work as a plasma researcher at the university, where he proposed the Helically Symmetric eXperiment (HSX) in 1992. HSX is a plasma fusion reactor using the stellarator design. However, this experiment utilizes supercomputer-optimized magnets to trick the plasma into thinking symmetric magnetic fields like those in a tokamak exist, a concept known as quasi-symmetry. HSX was “the first stellarator in the world that [gave] us confinement like a tokamak with the good engineering advantage of the stellarator”, Professor Anderson says, with the engineering advantage stemming from the elimination of the tokamak’s driven current. Construction began in 1993 and took six years to complete because of the complexities involved.
HSX was the first fully-optimized stellarator to test whether or not quasi-symmetry would work. Thanks to Professor Anderson and all those working on HSX, it has since provided significant scientific contributions to the designs of future stellarators. In essence, it proved that quasi-symmetric stellarators provide equivalent if not better particle confinement over tokamaks across multiple metrics. HSX was able to take a theoretical confinement technique and successfully demonstrate its effectiveness in practice.
With a key confinement problem solved, the next problem to solve is how to design more resilient reactors. “The biggest challenges facing fusion right now are materials. We have to deal with materials under extreme conditions,” Professor Anderson says. One of the reasons reactors would degrade is because of the current type of fusion reaction envisioned in the majority of fusion reactors around the world. This fusion reaction uses deuterium and tritium, two heavier isotopes of hydrogen. When these particles are fused, the output is a high-energy helium particle and, crucially, a high-energy neutron. Because neutrons are neutrally-charged particles and have no interaction with the reactor’s magnetic fields, neutrons formed in the reaction shoot out and can embed themselves into the wall of the reactor. Over time, this will cause the lining of the wall to degrade and exhibit some radioactivity. Even though the material would only be radioactive for a manageable 50–100 years — which is insignificant compared to the 10,000-year radioactivity of nuclear fission waste — it’s in the best interest of fusion researchers to figure out how to mitigate this high-energy neutron problem.
In Germany, a new experiment is beginning operation to test the viability of the stellarator as a reactor candidate. Wendelstein-7X (W7-X) is also a highly-optimized stellerator like HSX, but it will operate at larger energy levels and for longer time periods. Whereas HSX measures its operational timescales in milliseconds, W7-X will attempt to operate for thirty minutes continuously. Laurie Stephey, a graduate researcher with HSX capturing pictures of plasma and neutral particles to study their behavior, has seen the impressive nature of W7-X first-hand. In a complement to her work on HSX, she implemented particle imaging systems on W7-X to determine particle confinement durations. A core research goal of W7-X is the testing of a new type of device to sweep up the high-energy escaping plasma. This will allow W7-X to run for extremely long periods of time, which is critical to develop a grid-scale fusion reactor. “If W7-X is successful in this endeavor, it will make the stellarator a very attractive candidate for a future power plant,” Stephey says.
Energy generation is the ultimate goal of these two incredibly impressive research experiments. Nuclear fusion is truly the holy grail of energy production. To their credit, fission reactors can produce outstanding amounts of clean energy. However, fission reactors have highly radioactive waste and have the potential for creating large-scale disasters such as Chernobyl and Fukushima (it should be noted that modern fission reactor designs are significantly safer than those that melted down). Fusion reactors do not suffer from these radioactivity problems and do not have the potential to create a widespread nuclear disaster. As mentioned two paragraphs above, the most common fusion reaction uses deuterium and tritium. The fuel for this fusion reaction is abundant; one gallon of sea water contains enough deuterium fuel to match the energy output of at least 300 gallons of petroleum, and shooting the expelled neutrons from the fusion reaction into the element lithium produces more tritium. In contrast, the uranium for fission reactions must be excavated from mines and put through intensive purification processes. The heat from the fusion reaction will be used to boil water to spin a turbine to generate electricity, meaning the fusion reaction is carbon-neutral like nuclear fission. With readily-available fuel and an extremely clean reaction, it’s now up to our scientists to perfect the fusion reactor. This undertaking has been identified by the National Academy of Engineering as one of the Grand Challenges for the 21st Century.
Humanity has been researching fusion reactions for almost as long as we’ve been researching fission reactions. While it’s been a long-fought battle, we have never been closer to realizing widespread fusion energy generation. The implications will be far-reaching; the already-successful fission reaction can be replaced by a reaction that eliminates hazardous waste and is significantly safer to operate. Humans will cage miniature suns for our own benefit. Thanks to the fantastic work of David Anderson, Laurie Stephey and all those associated with HSX and the many other fusion experiments at UW-Madison and beyond, we are that much closer to realizing this reality.